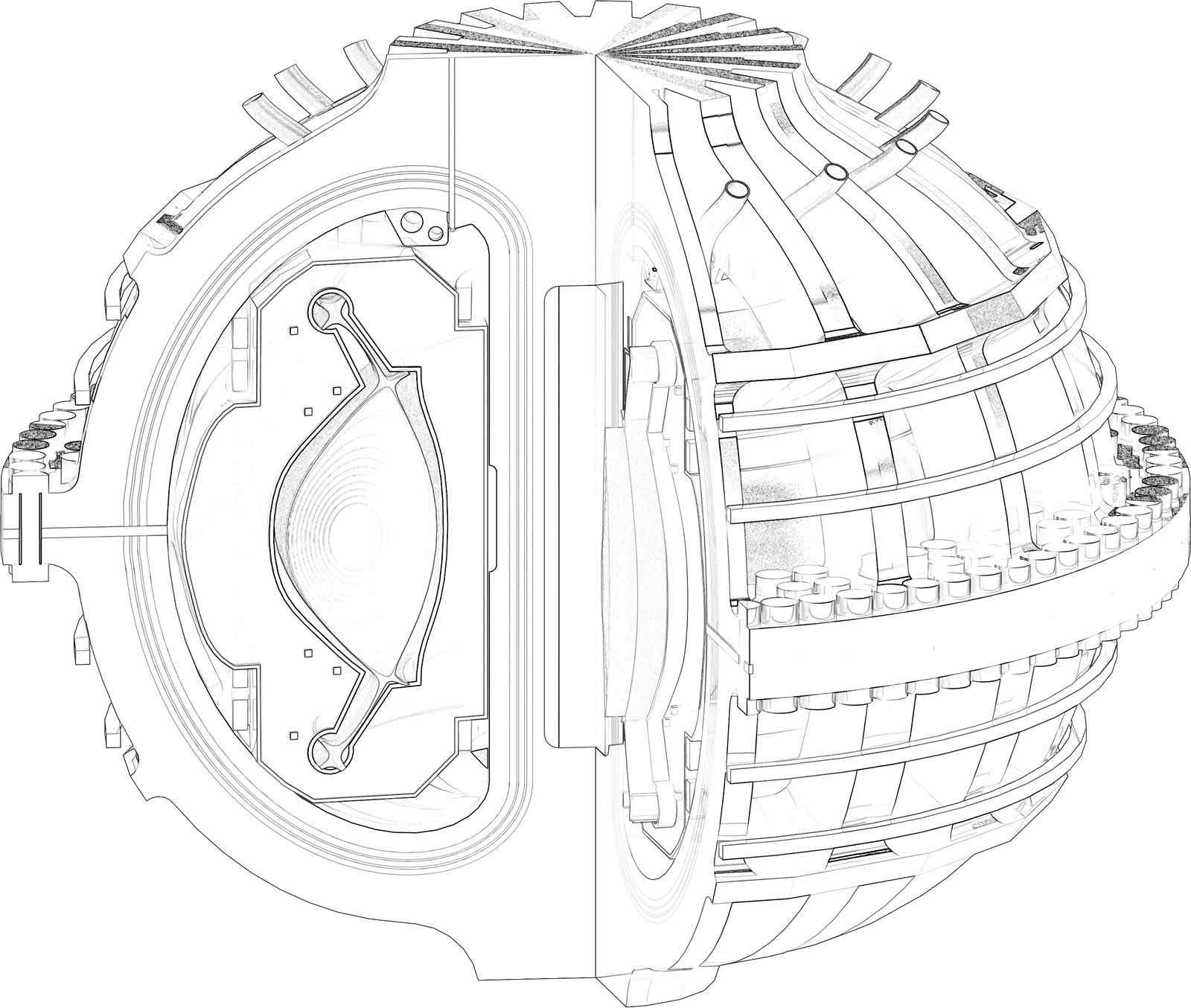
Engineering and Economic Challenges of Fusion
Fusion is generally touted by many as an energy "Holy Grail." Indeed, it appears to have similar qualities, being both perpetually elusive and miraculous, able to solve all mankind's problems. Media reporting tends to discuss the benefits of fusion with misleading and false statements and no discussion of fusion’s negative attributes. The financial and practical perspective of fusion based power is missing.
In general, fusion advocates have misunderstood or misrepresented the hard realities and complexities of deploying fusion power plants, potentially misleading the public into unprofitable investments. Policymakers, investors, and the public should assess the likely economics of fusion heat harvesting while sufficiently accounting for accident risk and spent fuel disposal. We aim here to add a dissenting perspective on the practicality of near-term fusion energy systems.
We view fusion not as a wildcard, but as a slowly unfolding engineering breakthrough with little practical utility to economically produce power or replace fossil fuels. MIT’s Dr. Lawrence Lidsky was a leader in fusion research in the 70s and 80s and was confident about its achievability. However, in 1984 he saw the challenges facing fission power systems and saw little future in the commercial deployment of fusion systems that failed to solve fission’s pressing problems, indeed augmenting the complexity and difficulty of economically producing nuclear power. “Long touted as an inexhaustible energy source for the next century, fusion as it is now being developed will almost certainly be too expensive and unreliable for commercial use.” [1] In the following discussion, we frequently bring up Lidsky’s observations, which have withstood 4 decades of fusion research.
The problem is cost.
The ultimate problem for fusion isn’t achieving ignition. It’s that fusion does not address the main impediments to wider scale deployment of current nuclear fission, primarily related to cost and megaproject risks. Fusion powerplants will be similarly sized to traditional fission power plants and far more complicated (ITER is $65B... other projects are in the $6B range). They will still be massive construction projects. At the end of the day, fusion is going to be the same one-off mega project construction project that the analysts criticize, with all its associated delays and cost overruns. This can't really be fixed without going to much smaller and simpler systems, and that's not really an option for fusion based on physical and engineering requirements of current fusion design concepts. The final section discusses the costs of fusion more fully.
The root cause is neutrons.
Operating a nuclear reactor, either fusion or fission, is a constant battle against the neutrons that damage and degrade reactor components. The great challenge for operating a fusion reactor is that fusion reactions produce 5-30 times more neutrons than fission reactions, and these neutrons have higher, more damaging energy. This excess of neutrons and neutron energy leads directly to immense radiation damage, significant radioactive waste, weapons material breeding capabilities, and significant bio shielding requirements. The knock-on effects are higher costs across the board — including high performance materials and coolants, high operating and maintenance costs, stringent security safeguards, high decommissioning, and waste disposal costs.
To examine the radiation damage potential of a nuclear power system, we examine the neutronicity of the reaction, which is the fraction of reaction energy released as neutrons. The higher the neutronicity, the higher the damage in the reactor structures. Table 2includes the neutronicity of various nuclear reactions, showing that Uranium fission has significantly lower neutronicity than fusion reactions pursued by leading fusion companies.
Most fusion concepts (Commonwealth Fusion, ITER) attempt D-T fusion because it has the lowest temperature ignition and is the easiest reaction to achieve. However, D-T fusions yields 32 x higher neutronicity compared to uranium fissions. D-He3 reactions, pursued by Helion Energy, require higher temperatures, and will include side reactions of D-D and D-T fusion, will end up having roughly 5-20x greater neutronicity than fission. The fusion reactions yield neutrons with higher energy that can travel meters and wreak havoc on reactor materials. On other hand, neutrons from fission are fewer and carry much less energy, stopping roughly 0.001 cm away from the reaction origin. Finally, p-B11 reactions can be reasonably considered aneutronic, or without neutrons, producing 25 times fewer neutrons than uranium fission. Still, even p-B has some side reactions among the reaction products that generate neutrons like B11+He4-3>N15+n+157keV. Unfortunately, p-B fusion requires much higher temperatures of 400 keV with significant Brehmstralung radiation that makes sustaining fusion reactions even more challenging. p-B fusion is not actively investigation by fusion research teams, except perhaps Tri-Alpha Energy.
Reaction | Reaction Energy [Mev] | Minimum Temp Required [K] | Neutronicity [fraction] | Relative to fission |
---|---|---|---|---|
D-T Fusion | 17.6 | 3x107 | 0.800 | 32 |
D-D Fusion (-> He3 or T) | 3.65 | 3x108 | 0.75. | 30 |
D-He3 Fusion | 18.3 | 6x108 | 0.050 | 2 |
p-B Fusion | 8.7 | 1010 | 0.001 | 0.04 |
Uranium Fission | 203.0 | No limits | 0.025 | 1 |
How do the additional neutron energy and availability affect the deployment of fusion systems? In short, the materials or systems to cost effectively survive the neutron damage do not yet exist, and the operational requirements and maintenance costs for dealing with such high radiation damage are likely to be cost-prohibitive. Radiation damage causes materials to swell and fracture, harden and embrittle, overall leading to greatly reduced performance properties. High material damage could mean rapid degradation of magnet performance, degraded thermal properties of the cooling fluids and surfaces, or embrittlement of pipes and pressure vessels. This is like running a car at higher than rated RPM. It can be done, but extra maintenance is needed.
In fission reactors, neutron energy deposition and damage are spread throughout the reactor core, and every effort is made to use the neutrons in the core without letting them escape. The opposite is true a fusion reactor where the neutrons need to be harvested in the blanket in order to breed fuel. Unlike the fuel elements in a fission reactor which can be easily replaced, the structural components of a fusion reactor are expected to be difficult to maintain or replace due to the high radioactivity of the irradiated components and because of the complex toroidal or other design with many interlaced and tightly packaged subsystems including the central solenoid, plasma vessel, first wall, divertor, vacuum gaps, neutron shielding, thermal shielding, magnets, and breeding blankets. Higher damage rates require more frequent replacement and maintenance, which requires the reactor to be shut down. The maintenance must be conducted without direct human actions due to the high radiation levels of the irradiated reactor components.
![Fig 2. From [2]. Overview of structural materials operational experience in a variety of civilian fission reactor systems compared with the proposed operating conditions for deuterium-tritium fusion reactors. ITER is a test reactor, not a power producing fusion facility, showing the massive disparity between fusion tests and fusion net power.](/assets/images/damage-1da39dd0d29a2267156060ccc4aeb8ed.png)
The above figure shows the operating temperature and radiation damage (shown as dpa or displacements per atom) that different nuclear power technologies must cope with. Fusion reactors would have to sustain radiation damage rates per energy produced an order of magnitude higher than current fission reactors and gas-cooled reactors, and somewhat higher than advanced fast fission reactors like TerraPower’s Natrium.
The figure also projects onto operational experience, revealing large deficits in data for fusion and fast fission reactor materials. Sufficient operational experience for new materials takes decades and is extremely valuable for understanding limitations and capabilities and fine-tuning material performance and applications.
![Fig 3. From [2]: 'The high neutron energy associated with the D-T fusion reaction generates approximately 50- to 100-times-higher He/dpa in materials such as ferritic steels than does fission reactor irradiation. ITER is a test reactor, not a power producing fusion facility.'](/img/fusion/damage2.png)
Below, we can see that high energy neutrons produced by fusion reactions and impinging on the reactor components will cause up to 100x the Helium deposition (leading to helium embrittlement) per atom displacement, which means the radiation damage caused by the fusion reactions includes much higher helium embrittlement relative to fission as well as the already discussed higher dpa. This leads to faster deterioration of properties and performance and requires high operation and maintenance costs.
Unfortunately, neutrons are not the only radiation source. Photon fluxes (counts/second/area) at the first wall are about 7 orders of magnitude greater for a D-T fusion system than the flux on an LWR reactor pressure vessel. These gammas are difficult to stop and require immense amounts of shielding material in the form of concrete, lead, and water.
No functional prototype.
Investors considering fusion must understand the outsize risk of investing in unproven technologies without working prototypes. How many fusion power plant projects are there? None, since the technology does not yet exist. The mining of asteroids is closer to commercial deployment than fusion plants providing power to the grid. On the other hand, fission is a mature technology, and some advanced reactors present few unknowns. The first fission reactor produced heat in 1942 and electrical power in 1951. Commercial deployments began in 1954, rising from less than 1GWe in 1960 to 100GWe in the late 70s.
This decades-long progression took place in the can-do Cold War era, with massive government investment and support, and a cowboy approach to R&D. Fusion reactors of any type are still chasing ignition, not even net-positive energy from the powerplant. After that Wright moment is achieved, perhaps in the next decade, challenges with commercialization and deployment are likely to be far worse than for fission power systems or other hardware systems given the order of magnitude greater technical and capital barriers to prototyping.
Other industries with historically massive product improvements and deployments have had much lower technical and prototyping barriers. Automobiles and aircraft are obvious examples. After the first demonstrations, there was an eruption in new companies commercializing car and aircraft designs. I remember reading somewhere that over 100,000 aircraft designs have been developed since the Wright brothers and on the order of 1000 commercial car companies have come and gone. It was relatively cheap to prototype and test designs and ideas, with a low regulatory burden and an eager societal embrace of the technology. Everyone and their mom had a new design. Even today, I could hack together a car or aircraft and test it on the road or in the air with little if any paperwork.
The technical and cost barrier for fusion devices means we are unlikely to see such an explosion of designs - especially in the West. The same would apply to fission systems because of a similar if not worse regulatory burden and cultural aversion. However, the cost barriers for developing a fission system can be 2-3 orders of magnitude lower than a fusion device, with few, if any, technical barriers. Just like cars and automobiles, there has been an explosion of new companies and fission design efforts in the last decade, though few have achieved significant hardware or licensing milestones highlighting the consequential cost and cultural barriers to nuclear demonstrations.
Some fusioneers claim there is fusion reactor operating experience at the dozen or so fusion research centers. These test reactors produce small amounts of fusion energy while drawing a much larger amount of grid power. They are roughly 2 orders of magnitude smaller in size and impact from a functioning fusion prototype with net electrical power output, and any licensing precedents from these research reactors can only be informative. Fusion reactors will be regulated by the NRC and will require a different but similar scale of licensing and regulation effort as fission regulations. These must still be deliberated and defined.[3] Fusion projects are a worthy cause no doubt, but not to be mistaken for near-term zero-carbon energy source, and certainly not at the expense of practical fission projects.
10x More Demanding Heat Transfer
Fusion reactors are 10x more demanding in heat transfer and 10x more demanding in thermal management.
By nature of the physical parameters required for fusion conditions, fusion reactors have a limited heat transfer surface requiring an extremely high heat transfer rate. For example, ITER will experience a sustained 10 MW/m2 on divertors, and 1 MW/m2 on the first wall, compared to a heat flux of 0.14 MW/m2 for USNC’s MMR. That is 10-100x less for the MMR.
Heat transfer technology that can also withstand high neutron radiation may not even exist to deal with such high heat loads. To sustain high heat transfer rates, the fusion devices will have to use more advanced cooling schemes and heat tolerant materials that will require more maintenance and cost, often introducing new challenges like chemical reactivity, corrosion, and activation that simpler technologies like the MMR completely bypass. These materials will also undergo extreme radiation damage, transmuting into other elements and requiring frequent replacement, as frequently as every 2-4 years of operations.
Thermal management in a fusion reactor is unusually challenging because of the extreme temperature gradients, multiple vacuum gaps, as well as temperature changes and cycling which can be dramatic when pulsing. There are 100 million degree plasmas, 600-1000 °C coolants, -40 °C super magnets — all within a few meters. And all the materials are heavily irradiated by high-energy neutrons and gammas. This is well beyond the engineering challenges faced in space, and commercialization would take significant time and effort.
Parasites: 1.5-2x more generating capacity
Parasitic Power Drains require 1.5-2x more electrical power generating capacity. How frustrating it will be to work at a fusion powerplant, where half or more of the electrical power produced must be wasted at the site. Electrical energy must be generated and used to create fusion conditions and support the powerplant. For every MWe produced by a fusion powerplant, half or more has to be used by the fusion powerplant. No fusion device has generated electricity, but the best fusion prototypes have achieved a roughly 1:3 ratio of thermal power produced to electrical power consumed (~1:9 in electrical terms), and the stated estimates usually only include the power directly pumped into the plasma, omitting the immense power to operate the powerplant (see ITER’s layout in Figure 9) which includes cryocoolers, tritium processing, and vacuum pumps.
These auxiliary power needs favor economies of scale towards very large powerplants, with estimates suggesting that fusion powerplants at or below 300 MWth, would not be able to supply enough power to cover the powerplants’ own electrical consumption.
Parasitic power drains are an immense drawback as a fusion-powered grid would require on the order of 1.5 to 2 times the electrical power generating equipment and coolant water supply compared to a fission-powered grid. The number is reduced as the fusion plant becomes larger. The extra electrical power is used to power the fusion reactors. This immediately translates into a multiplier on the material and operating cost for generating a unit of electrical power and an extra hurdle for making fusion an economical power source.
High Temperature Reactor like MMR have a thermal to electrical efficiency will start out at a modest 33%, similar to traditional nuclear power stations, and will increase to 45-50% as custom-built turbines are incorporated into the design, and higher temperatures are accessed for more efficient power conversion. This is an exactly inverted trend compared to fusion
50-100x more radiological waste volume
Radiological waste production from fusion power plants would be 50-100x larger by volume than fission for the same power produced.
While fusion systems do not produce the same long-lived waste as fission, the abundance of energetic neutrons and gammas in the fusion process requires immense shielding and causes activation of the structural components of the reactor which must then be classified as both low-level waste (LLW) and intermediate-level waste (ILW) . From IAEA, "The activation of the reactor's structural material by intense neutron fluxes is another issue. This strongly depends on what solution for the blanket and other structures has been adopted, and its reduction is an important challenge for future fusion experiments." An example of the immense quantities of shielding materials can be found in this report.
"Under current UK nuclear law, ILW requires geological disposal. Therefore, the latest research indicates that fusion plants could produce nuclear waste which requires long-term subsurface disposal, similar to fission plants. Although fusion won't produce high-level waste (which requires active cooling) and also won't produce radioisotopes with half-lives greater than 100,000 years, the need for deep geological disposal still weakens one of the main arguments for fusion over fission. It is hard to avoid this: the elements responsible are either required for mechanical properties or are present as natural ore impurities in the tens of parts-per-million concentrations, the reduction of which might not be technically or economically feasible."
![Fig 4. Waste volumes from several fusion system designs compared to a fission boiling water reactor, from [6a]. The waste volumes are not normalized for the net electrical energy produced by the given power system. Indeed, the ESBWR will probably produce an order of magnitude more electrical power than any of the systems listed. Furthermore, the estimates do not include the maintenance and replacement parts for fusion subsystems are heavily irradiated and will have to be replaced on a yearly or every couple year basis.](/assets/images/waste2-b400509b107010d5c2b0cca04e31673a.png)
The fact that a fusion reactor does not produce long-lived fission products in the fuel like fission, would seem a clear advantage compared to fission. But in practice, there is no cost advantage. Indeed, the much larger irradiated waste streams from a fusion power plant will likely yield greater decommissioning and waste disposal costs compared to fission. Figure 4 compares the waste volumes of several fusion test reactors and the ESBWR design from GE-Hitachi. The plotted data does not normalize for net electrical energy produced over the lifetime of the plant as none of the fusion power systems in the study produce net electricity.
We compute an estimate of energy normalized waste volumes using design papers for commercial fusion designs, such as ARC [5] or DEMO, and their reported component lifetimes (ranging from 4-10 years) and volumes. For the fission ESBWR, we use the 60-year lifetime and known waste generation rate. The simple calculation suggests fusion power systems will produce on the order of 100 times more radiological waste volume per unit of electrical power delivered. The calculation included recycling and optimistic capacity factors and reactor lifetimes.
Fission yields tiny volumes of long-lived waste while fusion produces very large volumes of short-lived waste. It is not clear whether one is better than the other, but smaller volume problems tend to be more manageable at lower cost. Some fusion waste considerations are listed below.[6a][7]
- Large volume to be disposed of (7000--8000 m3 / GW
eplant including bioshield and not accounting for part replacement over the lifetime of the plant). - High disposal cost (for preparation, packaging, transportation, licensing, and disposal).
- Limited capacity of existing LLW repositories.
- Need for fusion-specific repositories designed for T containing radwaste.
- Need for specific activity limits for fusion LLW issued by legal authorities.
- Political difficulty of building new repositories.
- Tighter environmental controls.
- Radwaste burden for future generations.
Financial Risk of Powerplant Loss
Like any industrial equipment, fusion reactors can be subject to accidents and destruction of the powerplant.
We often hear from policymakers, media, and scientists that, for fusion "nuclear accidents can't occur in the same way as they do for nuclear fission." This is misleading. Viewed from the perspective of risk to investment, we expect fusion powerplants to pose higher risk of investment loss compared to traditional fission power systems, and a comparable risk compared to fission nuclear plants under development today.
It is true that fusion reactions will stop after a few seconds if the reactor is properly shut down or goes out of normal operating conditions, primarily because the fusion conditions are so difficult to maintain in the first place. This inherent safety claimed by fusion systems is equivalent to the inherent safety achieved by the USNC Micro Modular Reactor (MMR), except the MMR has no complex fuel breeding systems or magnets and no chemical risks associated with the breeding fluid (FLiBe).
The MMR really is an unusual system, even for advanced reactors and micro reactors. The main difference is the astoundingly low power density. The entire 3x8m reactor pill produces the same power as a single LWR fuel bundle, which measures a little over a meter.
As in a fusion system, the MMR simply stops producing heat from nuclear reactions, without any action or mechanism, when conditions deviate from the operating conditions. MMR and fusion safety are both fundamentally different from other fission reactors that must be carefully shut down while maintaining fluid cooling capability to the reactor core to prevent meltdown. Just like fusion, the conditions to maintain power production in MMR are difficult to maintain, and deviating from those conditions by heating the reactor core or losing cooling ability brings reactions to a halt. Any remaining heat in the core is removed by solid-state processes like conduction and radiation -- no moving parts or fluids.
Now, the accident possibilities that lead to damage or destruction of fusion systems have yet to be fully explored, but the complexity and high performance/bleeding edge nature of a fusion system suggest a fertile ground for mishaps that can cause a total or partial loss of the fusion device. In other words, the physical conditions, stored energy, and system complexity involved in a fusion device, suggest there are many event trees that can lead to a disabled or destroyed fusion device and loss of financial investment. Where the MMR deviates from the fusion systems is its much lower system complexity as quantified by order of magnitude lower part counts, less than half the subsystems, and a strong operating experience which has direct effects on capital and operating costs.
As one example of the system complexity, the heat fluxes in fusion devices are much higher than for the MMR by 10-100x depending on location in the device. The need for active cooling systems in fusion devices is paramount as loss of coolant can quickly lead to a damaged device even when the reactor shuts down.
Decay heat is the extra heat produced after a reactor is shut down from radioactive decay of fission products. Failure to remove this heat is what leads to reactor reactor melt-downs. We note that, like fission systems, fusion devices contain significant decay heat in the irradiated structural materials starting at 2.7% of fusion power, compared to 6.6% for normal fission systems, which still requires careful thermal management and design to avoid catastrophic system damage during system malfunctions. Decay heat profiles are show below.
Back to the MMR example. MMR has a lower decay heat power density than fusion systems like SPARC or ARC, DEMO, or ITER and orders of magnitude lower than other advanced fission reactors as show in the figure below. UNSC's MMR has the lowest decay heat power density at 0.075 W/cm3, less than DEMO's 0.083 W/cm3 in the blanket and divertor. A lower decay heat is more manageable by passive cooling systems, allowing the reactor to dissipate heat more easily and without damaging the reactor. The other aspect to consider is the maximum temperatures that can be safely maintained in the reactor. Gas-cooled reactors like the MMR have all-ceramic cores that can withstand much higher temperatures than a fusion's reactors metals, molten salts, and magnets. MMR's low power density is a paradigm shift in nuclear safety, more foundational than fusion, for it can be accomplished cost effectively today.
The above decay heat comparison is for the activated components of a fusion reactor, but a similar comparison can be made to the decay heat in the breeding blanket [6b]. It should be noted that smaller fusion systems will have greater decay heat power density than ITER or DEMO.
Another risk for Tokomaks, is the possibility of superconducting magnets going normal which could occur with unexpected power changes. When this occurs, the magnets can explode in a physical sense -- an exothermic event where the density suddenly changes, and the physical containment fails, causing large-scale financial damage to the powerplant. Operating a fusion powerplant carries similar risk to a traditional nuclear powerplant like Three-Mile Island, where an accident can cause destruction of the powerplant and total loss of investment without leading to a radiological release - at least if there's a containment structure, which many fusion designs would like to omit.
We've illustrated the different financial risk profiles in the figure below to compare traditional nuclear, fusion, and USNC's MMR. Nuclear is already statistically safe with a low number of directly attributed deaths in its six-decade history. But significant property and financial damage have been caused during black swan events as seen in Japan. Because of the high fission product inventory combined with the high power operation of many fission reactors, fission has had the highest probability of catastrophic and expensive accidents (>$1B
) with damage extending beyond the site. Fusion, overall, has less risk of >$10B
accidents because there are far fewer fission products to release during an explosive accident or meltdown, though the release of Tritium and irradiated structural materials still presents a risk. But fusion has a greater risk for <$1B
events due to the relative complexity of fusion systems.
Fusion complexity arises simply due to the larger number of systems and coordination between them, many of which are totally absent in a fission system or are more challenging to implement. There are magnetic fields and plasma heating systems, vacuum pumps, extremely large vacuum vessels, and an entire system for breeding fuel online. Compared to fission, fusion will require breakthroughs in high-performance materials and components that will experience unprecedented radiation damage, record temperature gradients, 10-100x thermal fluxes. Accidents and malfunctions are simply more likely for more complicated systems because more things can go wrong. And unfortunately, even small repairs to a fusion device will be extremely costly because of the radioactivity of the activated components. "From a manager's perspective, all reactor systems that are too radioactive for hands-on maintenance are equivalent: if something major breaks, it is unrepairable."[1]
Fusion may be the most expensive way to generate carbon-free power in the next 4 decades
Analysts tend to correctly identify some of the challenges with traditional nuclear fission reactors associated with high capital costs, megaproject cost and timeline slip (example). Unfortunately, fusion systems offer no solutions to the cost and schedule problems that have weighed down fission power systems -- there is no SMR or MMR approach in fusion. Indeed, the problems with (traditional) fission are exacerbated because fusion power plants must be built big, at high power, with 1.5 to 2x the electrical power conversion and cooling equipment compared to fission systems and requiring more numerous and more complex subsystems that are more difficult to maintain and are damaged more quickly. This translates into higher capital, operating, and decommissioning costs. And all that for less net power produced and more volume of total activated waste.
Fusion devices using tokamak designs will likely remain quite large as either magnetic confinement limits, thermal hydraulic, or material limits are reached. This means that D-T fusion devices will likely always remain large reactors in the 300+ MWth range. Even at 3 meters, the initial and no doubt starry-eyed capital cost estimate for the 525 MWth ARC reactor comes in at $5.5B excluding the balance of plant and bio-shield (e.g., 3 meters of nuclear concrete), as shown in the table below.[5] The high radiation damage means that many of these high capital cost items such as the first wall, vacuum chambers, and FLiBe tank and fluid will be replicated as O&M costs every 4 to 10 years.
A fusion powerplant will inherit most of the systems present in a fusion power plant including security and safeguards, power generating equipment, and activated material storage areas. It will also have additional systems required for fusion devices: the magnets and heaters to maintain fusion reactions, cryocoolers and vacuum pumps for the same, tritium breeding and refueling, robotic equipment to support high intensity maintenance schedule, sealed areas and equipment to deal with Tritium and heavily irradiated components, and massive bio shields for the high neutron and gamma radiation. Beyond the capital cost increases, all these additional subsystems and operating activities can be expected to more than double the personnel requirements compared to a fission system.
Overall, fusion devices retain the nuclear complexity of a fission plant and then add complexity and cost with new systems and high-performance materials for fuel breeding, cryo-cooled superconducting magnets, extremely high heat fluxes, and extreme radiation damage.
Fusion powerplants will be using the bleeding edge in high-performance materials, including the highest performing superconducting magnets, FLiBE for breeding fuel and cooling the reactor, and the most radiation-resistant steels, metals, and ceramics - many of which need to be newly invented. A fusion reactor will be the ultimate in high performance and high-cost systems, the Bugatti Veyron of energy production, requiring greater capital costs, double or more the operating and maintenance costs, and producing 10-100x the waste volume compared to a fission system.
In contrast, reactors like the MMR are more like a Corolla, mass-produced using standard off-the-shelf and proven materials, at modest capital cost, minimized operating costs with as much automation as possible. Simplicity, low cost, safety, and reliability go hand and hand, and are the 4-in-1 bundle needed for massively deployed hardware products.
Different but significant proliferation risk
We often hear that "fusion reactors cannot be used to produce weapons materials." This is simply not true - fusion system are specifically designed to breed new nuclear materials as part of their fuel cycle.
All nuclear systems must account for the possibility that nuclear material can be used to produce nuclear weapons. The focus in non-proliferation efforts has been to prevent the diversion of U-235, Pu-239, and U-233, which can be used for nuclear weapons even in small amounts of 1 kg. Fission reactors use and produce these materials and are generally safeguarded by strict federal and international material handling and monitoring protocols which have proven effective over the last six decades. Proliferation is further mitigated by the fact that the spent fuel contains a high fraction of non-weapons Pu-240, which makes it difficult to use in a weapon, and is mixed with dozens of other radioactive fission products and isotopes that require sophisticated and costly chemical processing techniques to extract the materials efficiently and safely.
Many advanced reactors use TRISO particle fuel. TRISO fuel enhances non-proliferation efforts by 1) achieving high fuel burnup thus lowering the quality of weapons materials, 2) avoiding fuel reprocessing altogether, and 3) encapsulating fuel at the millimeter scale in multilayered refractory ceramics, making the extraction of weapons materials extremely difficult if not impossible.
As previously discussed, fusion reactors produce an excess of neutrons that must be utilized for breeding new fuel and are therefore capable of producing weapons materials. Indeed, the blanket surrounding the reactor is specifically designed to breed nuclear materials and can, with reasonable effort (and breaking of international safeguards law), be repurposed to produce significant and pure quantities of weapons material at rates similar to fission breeder reactors.[10] Fertile material like U-238 or Th-232 could be introduced into the breeding blanket or anywhere in the reactor chamber with sufficient neutron flux and become transmuted to weapons-grade Pu-239 or U-233.
On a separate note, the Tritium generated in a fusion reactor to fuel the D-T reactions can be used to "boost" warheads and lower the amount of fissile material required to make a weapon. There is no exaggeration here. One warhead requires roughly 4 grams of Tritium, and in 1995, the US's 10,000 warhead arsenal required just 2.2 kg/year for Tritium replenishment.[11] While only a few grams of Tritium will be in the plasma chamber at any given time, a fusion powerplant may be expected to hold between 1 and 4 kg of tritium inventory. Current worldwide demand for Tritium for lighting and watches is under 400 grams per year.
These material creation and diversion possibilities are almost comically simple and could be difficult to detect at low production rates as they do not require any special input materials. Fission reactors do not have the same ability to conveniently generate weapons material outside of the fuel because there is 30 times less neutron energy available, and all the neutrons are carefully used to initiate new fission reactions in the reactor core rather than breeding Tritium in a breeding blanket. Certainly, fusion powerplant safeguards can be implemented to prevent the misuse of fusion neutrons or fusion fuels, but these will require a similar non-proliferation effort as currently required for fission.
Unclear Motivation
The original driving force behind fusion reactors was the promise of abundant fuel, not lower accident consequences or reduced cost. Fissile elements for nuclear fuel (Uranium resources) were supposed to be very rare. We were going to run out of nuclear fuel in just a few centuries, and so we needed fusion reactors or fast reactors with high breeding ratios to meet growing energy needs. Both assumptions are outdated and mistaken.[12] Known deposits, including the ocean, provide nearly inexhaustible amounts of fission fuel. Reactors with conversion ratios below 1 like current light water reactors and many advanced reactors can be sustained for many thousands of years. The abundance of Uranium also makes Thorium fuel cycles unnecessary in the short run. Even so, many advanced reactors can use Thorium just as effectively as Uranium.
It's pretty clear fission fuel costs or availability are not a concern and no longer provide grounds for fusion development. Compared to fission reactors like MMR, fusion systems have no advantages in terms of safety, waste, or cost. Indeed, the foregoing discussion suggests higher financial risk, more burdensome waste management, and a higher cost of energy.
So what is the motivation? Fusion projects funded by billionaires, VCs, and investment banks appear to possess authentically held commercial ambitions. Maybe they know something I don't. Or perhaps the investments are more like research donations or tax write-offs. It's a good tax strategy, far better the deranged modern-art market by a long shot. And it's probably more efficient for to send money directly to promethean R&D projects instead of routing the cash through the grasping hands of the IRS, congress, and bloated government departments all to be misappropriated by some unelected, second-rate bureaucrat.
Government fusion projects have strong non-commercial motivations, funding lots of research in materials, plasma physics, and engineering to support the demonstration. ITER may also have a non-proliferation justification, keeping European and Soviet talent tied up in research instead of weapons.
Hype = Financing
Fusion proponents, aided by media, have painted a commercial vision which they cannot realistically deliver in the next three to four decades. Is hype the only way they can obtain financing? They perpetually over promise to investors and the public, successfully diverting financing and support from other nuclear energy projects. I reproduced an expanded version of Jassby's fusion hype list [13] below. All the targeted milestones below have yet to be completed as of 2022. While a bit gloomy, the list is a monument to human optimism and determination. But it's also a warning to investors and the public.
All the fusion financing and hype is fine as long as practical energy solutions are also funded and implemented. But I have a feeling that fusion promises are diverting the speculative and commercial investment that would have gone to advanced fission. Why would anyone finance new fission designs and projects that will last 40 years when holy-grail fusion reactors will soon be widely available? Perhaps this is changing as corporate and national decarbonization promises start to meet practical realities.
Jassby's Hype List
ITER
The ongoing ITER prototype, has blown 4x over budget to $65B with 2x schedule delay to mid 2030s.
General Fusion (GF)
"GF believes it can build a grid-capable fusion reactor rated at 100 megawatts four years later for about $500 million, beating ITER by about 20 years and at a fraction of the cost." MIT Tech Review, July 2009.
"GF targets prototype by 2015 and a working reactor by 2020," from NBF 5/19/2012.
"GF will demonstrate DD-equiv. net (energy) gain in 2016," and "GF targeting commercial reactor for 2020," from NBF 5/24/2013.\ GF will demonstrate net gain in 2018 and
"GF targeting commercial reactor for 2023," from NBF 8/18/2015.
"GF Demo nuclear fusion plant around 2023", quoting C. Mowry, CEO of GF, from NBF 5/23/2018.
Helion Energy
"Helion's cost of electricity production is projected to be $0.01 per kWh without assuming any economies of scale from mass production, carbon credits, or government incentives." From Helion's site 11/2021.
"The Helion Fusion Engine will enable profitable fusion energy in 2019," from NBF 7/18/2014.
"If our physics holds, we hope to reach that goal (net energy gain) in the next three years," D. Kirtley, CEO of Helion, told The Wall Street Journal in 2014.
"Helion will demonstrate net energy gain within 24 months, and 50-MWe pilot plant by 2019," from NBF 8/18/2015.
"Helion will attain net energy output within a couple of years and commercial power in 6 years," Science News 1/27/2016.
"Helion plans to reach breakeven energy generation in less than three years, nearly ten times faster than ITER," from NBF 10/1/2018.
"We expect that Polaris will be able to demonstrate the production a small amount of net electricity by 2024. We will continue to iterate our device quickly so we can offer commercial fusion power for the grid as soon as possible." From Helion's site 11/2021.
Lockheed-Martin Compact Fusion
"Lockheed will have a small fusion reactor prototype (powerplant) in five years...and a commercial application within a decade," from MIT Technology Review, 10/20/2014.
"Net energy gain in 2020 and commercial powerplant targeted for 2024," from NBF May 3, 2016.
Fuse Energy Technologies
Tokamak Energy
Zap Energy
Focused Energy
Xcimer Energy Energy
- Phase One: A prototype laser system in the Denver facility within the next two years
- Phase Two: A full-scale engineering breakeven demonstration facility at some point after the first phase
- Phase Three: A fusion pilot plant within ten years
Tri-Alpha Energy (now TAE Technologies)
"Tri Alpha says it will produce a working commercial reactor between 2015 and 2020," from NBF 8/16/2011.
"Tri Alpha Energy now likely 2020 - 2025..... for commercial nuclear fusion," from NBF 10/16/2015.
"Tri-Alpha Fusion to develop commercial fusion by 2027," from NBF 1/19/2017."
The company will generate net energy from fusion.... in about five or six years," from K. Bourzac [8], 8/6/2018.
TAE once planned to exploit the aneutronic p-11B reaction, but tacitly abandoned that goal when it dropped "Tri-Alpha" from the company name.
Commonwealth Fusion Systems
"Massachusetts Institute of Technology has announced that its SPARC reactor could begin producing energy from nuclear fusion by 2025", Power Tech 2019.
First Light Fusion
"The startup claims it is on target to achieve fusion gain (more energy out than goes in) by 2024.", eeNews 2019
Lawrenceville Plasma Physics "founded in 2003, has been living crowdfund-to-mouth since 2014 when it raised about $200,000 with the promise of a working fusion machine by the year 2020. Its latest "stock" sale, which happened a few months ago, raised more than $2.2 million, while suggesting to investors that it would have a functional prototype within three years."
Conclusions
Discussions about fusion are dangerously misleading for the public, investors, and policymakers. Fusion reactors are not likely to be available for decades and cannot be expected to compete with any power source in the near term on simple physical and economic grounds. No fusion approach today can get rid of the neutrons. Fusion’s high neutron production will generate massive quantities of radioactive waste which compares with fission in total diluted toxicity for the first 10,000 years. The suggestion that fusion reactors cannot have financially destructive accidents like Three Mile Island is wrong. The economics are not promising. Fusion does not seem poised to solve the waste, safety, or cost concerns associated with conventional fission reactors.
There is a cost to the fusion hype in that it distracts financing and willpower from practical solutions available today. It allows people to think that a future idealized solution will solve all of today's problems instead of immediately using readily available solutions. We already have the tools for reliable and zero-carbon energy without increasing consumer costs and power outages. We don’t need fusion wild cards. We just need the resolve to quickly manufacture fission reactors for planet-scale deployment. In that zero-carbon fission-based civilization, fusion systems may finally come of age.
Further Reading
- Lidsky, “The Trouble With Fusion.”
- Zinkle and Snead, “Designing Radiation Resistance in Materials for Fusion Energy.”
- White, White, and Whyte, “Regulatory Frameworks and Evaluation Methodologies for the Licensing of Commercial Fusion Reactors By.”
- Nicholas et al., “Re-Examining the Role of Nuclear Fusion in a Renewables-Based Energy Mix.”
- Sorbom et al., “ARC: A Compact, High-Field, Fusion Nuclear Science Facility and Demonstration Power Plant with Demountable Magnets.”
- a. Pohorecki et al., “Activation, Decay Heat and Waste Analysis for a European HCLL DEMO Concept.” b . Eade et al., “Activation and decay heat analysis of the European DEMO blanket concept.”
- Gilbert et al., “Waste Assessment of European DEMO Fusion Reactor Designs.”
- Gilbert et al., “Activation, Decay Heat, and Waste Classification Studies of the European DEMO Concept.”
- El-Guebaly et al., “Goals, Challenges, and Successes of Managing Fusion Activated Materials.”
- Goldston and Glaser, “Safeguard Requirements for Fusion Power Plants.”
- Kalinowski and Colschen, “International Control of Tritium to Prevent Horizontal Proliferation and to Foster Nuclear Disarmament.”
- MIT, “THE FUTURE OF THE NUCLEAR FUEL CYCLE.”
- Jassby, “Voodoo Fusion Energy.” and Jassby, The Quest for Fusion Energy
Originally written with USNC to respond to a misguided Morgan Stanley report on the prospects of nuclear energy.