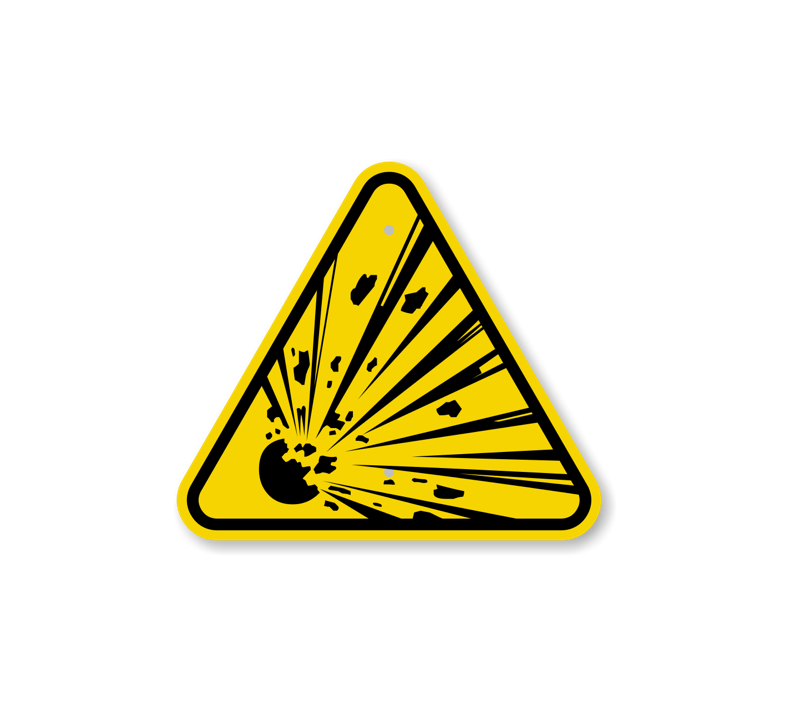
Hazards for Nuclear Reactors
Hazards and Mitigating Design Choices
A nuclear reactor contains many hazards that can ultimately lead to a Fission Product (FP) release including decay heat, excess reactivity, chemical reactions, and high pressure. Hazards can be considered the potential gradients that can or could become accessible and who equilibration can lead to fission product release.
The fission products in the fuel are the underlying hazard. Fission product gases are concentrated in the fuel at high pressure and temperature, but nonexistent in the low temperature and low-pressure environment. This form a chemical difference that would like to equilibrate to the disadvantage of lifeforms outside.
Each hazard is listed in the table below with the mitigating approach taken in the HTGR architecture. Each in their own, the hazards can be mitigated through passive mechanisms and inherent characteristics. The International Atomic Energy Agency’s (IAEA) definition of inherent safety being “safety achieved by the elimination of a specified hazard by means of the choice of material and design concept” and their logic on the various categories of passive systems is followed.1 The second Table discusses various design choices, and their effects on the hazards.
HAZARD | Description |
---|---|
Chemical Hazard | Core components can interact with each other, the coolant, or external water and air to release energy or cause corrosion. At a minimum, this exacerbates maintenance and inspection needs during normal operations. During accidents, these features escalate conditions beyond the point of no return such as when Zirconium and water react at elevated temperatures to release energy and hydrogen which can then explode resulting in loss of control functions or LOCA. Similar problems occur with sodium coolant and heat pipes. |
Reactivity Hazard | Control rod extraction or other mechanisms can cause the reactor to become dangerously supercritical and dramatically increase the power output of the reactor beyond what it is capable of handling. Too much power and the fuel will melt itself, releasing fission products. Most reactors can somewhat tolerate the partial ejection of one or two control rods but will fail catastrophically when more are extracted. |
Terrorist Hazard | Bad actors may intentionally sabotage reactor components by explosive or kinetic means. Bad actors could intentionally withdraw all reactor control rods, cause intentional coolant depressurization or flooding. |
Pressure Hazard | High pressure constitutes a stored potential energy that can be released explosively leading to impacts and damage to reactor components or the release of radioactive coolant. Pressure can escalate accidents. |
Decay Heat Hazard | When a reactor shuts down, it continues to produce some fraction of the power as decay heat for hours and days. This decay heat is what leads to reactor melting, when the reactor operators are unable to cool the reactor down, such as in the case of pipe clogging, loss of power for pumps, or loss of coolant. |
According to several studies, a TRISO fueled HTGR reactor is the highest TRL Generation IV technology 2, the most able to mitigate chemical and reactivity insertion hazards, and has sufficient acceptance from regulators and nuclear skeptics 3. This perspective is becoming mainstream in academic 4 and industrial circles with HTGR being the most heavily invested advanced reactor technology besides LWR SMRs. It is postulated that this class of reactors can be characterized by small unit power systems connected to a large balance of plant, with few or no safety systems or safety grade equipment and lower operating costs. For HTGRs, this risk reduction is principally achieved by limiting power rating and using refractory ceramic materials so that fuel temperatures do not exceed limiting temperatures during simultaneous Beyond Design Basis Accidents (BDBA), assuming an appropriate degree of security and safeguards is provided to the reactor, and all while using only Class A Passive safety mechanisms. This reactor technology is called the Class A HTGR (CA-HTGR), which more or less mirrors the design architecture of the commercially developed USNC MMR.
Hazard | HTGR Approach |
---|---|
Chemical Hazard |
|
Reactivity Hazard |
|
Terrorist Hazard |
|
Pressure Hazard |
|
Decay Heat Hazard |
|