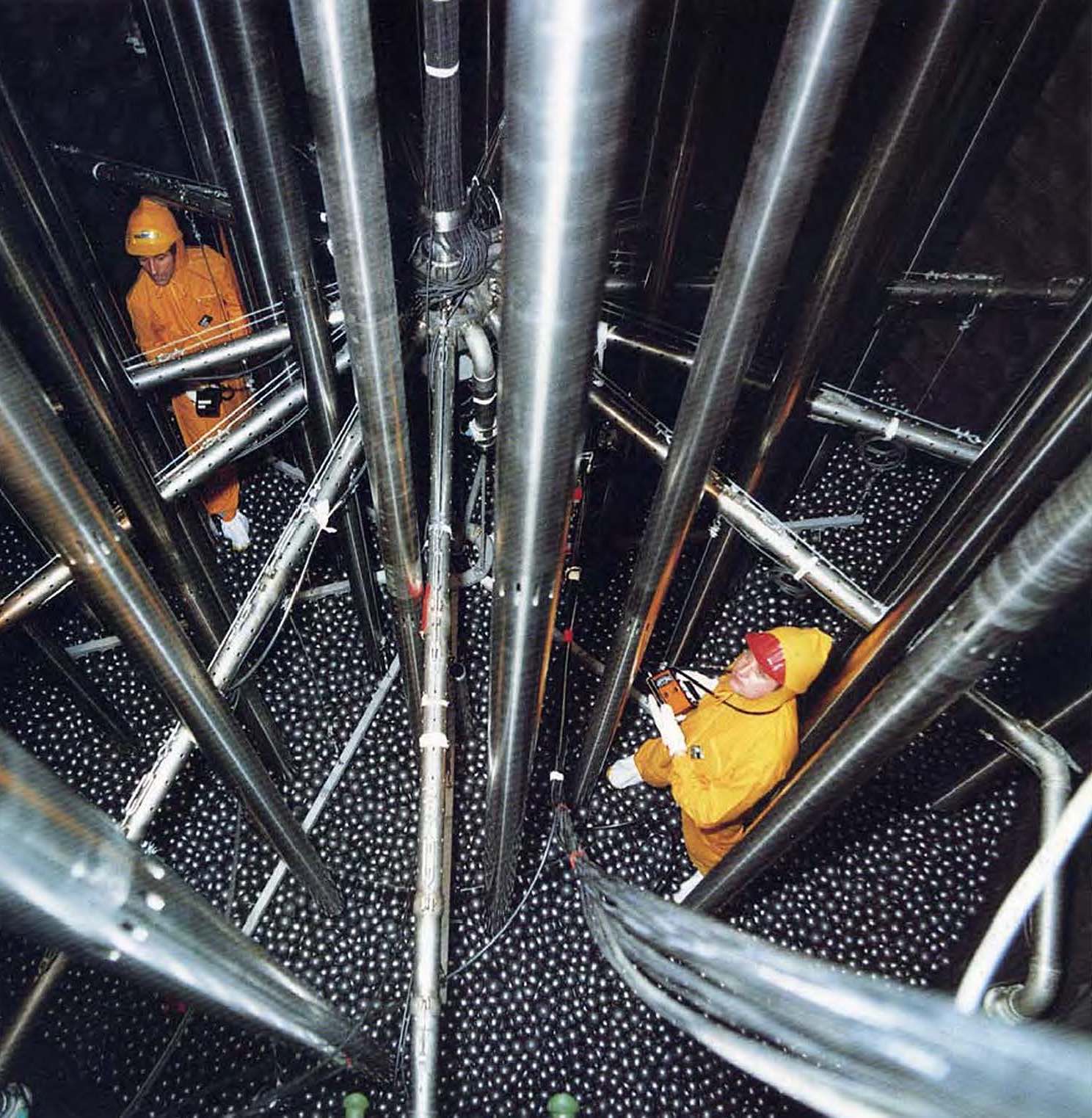
Pebble Bed - The Nuclear Gumball Machine
2024/10/16 Note: since I wrote this in 2022, Google and Amazon have decided to invest in the technology and development of pebble bed TRISO-fueled reactors with Kairos Power and X-Energy. They must have forgotten to click the subscribe button on my newsletter! I predict both these projects will be completed but neither will be pursued for larger gigawatt scale rollouts without dramatic design changes approaching prismatic HTGR.
High temperature gas-cooled reactors (HTGR) come in basically two flavors: prismatic cores and pebble-bed cores. Both use graphite moderator and TRISO fuel particles to make the core, which allows the reactor to reach high temperatures and handle accidents with relative ease. Both use helium to cool it, which allows thermal applications up to 950°C and power conversion efficiencies of 40%-55%, compared to just 300°C and 30% in water-cooled reactors. They differ in the geometry and form factor of the moderator and fuel, which leads to significant differences in size, operations, and technology roadmaps.
In prismatic cores like the Ultra Safe MMR or Japan's HTTR, graphite is formed into chair-sized hexagonal blocks with holes for cylindrical fuel pellets and separate holes for coolant channels. The core is maximally packed and nothing moves. Refueling the reactor involves swapping out the graphite blocks.
In Pebble Beds like China's HTR-PM or the very similar X-Energy Xe-100 design, the fuel and graphite are packaged as balls (called pebbles) that are poured into the reactor from the top and emptied at the bottom in a continuous fashion like a gumball machine. The basic working principle of a pebble-bed is shown below.
This post is primarily about pebble-beds but provides the needed comparison to prismatic HTGRs to illustrate the benefits or drawbacks. For the most part, I focus on the drawbacks that are unique to pebble-beds as most of the benefits apply to both prismatic and pebble-bed reactors.
Quick Summary
Compared to prismatic cores, pebble beds introduce several drawbacks as summarized below:
- Challenging fuel handling problem with extra infrastructure → lousy capacity factor.
- Pebble cracking and jamming leads to shutdowns and difficult unjamming
- Worse proliferation/diversion profile
- Single-use moderator = more waste volume and cost
- 1-dimensional un-optimizable cores, waste 30-40% of the core volume so they have to be big and inefficient, while still maintaining a high pressure boundary
- Extra stress on the fuel with jostling and core weight
- Produce more moderator waste
- Pebble release failure mode
- Dust generation and fire/energy hazard
- Dead-end designs that are not set up for changes or improvements
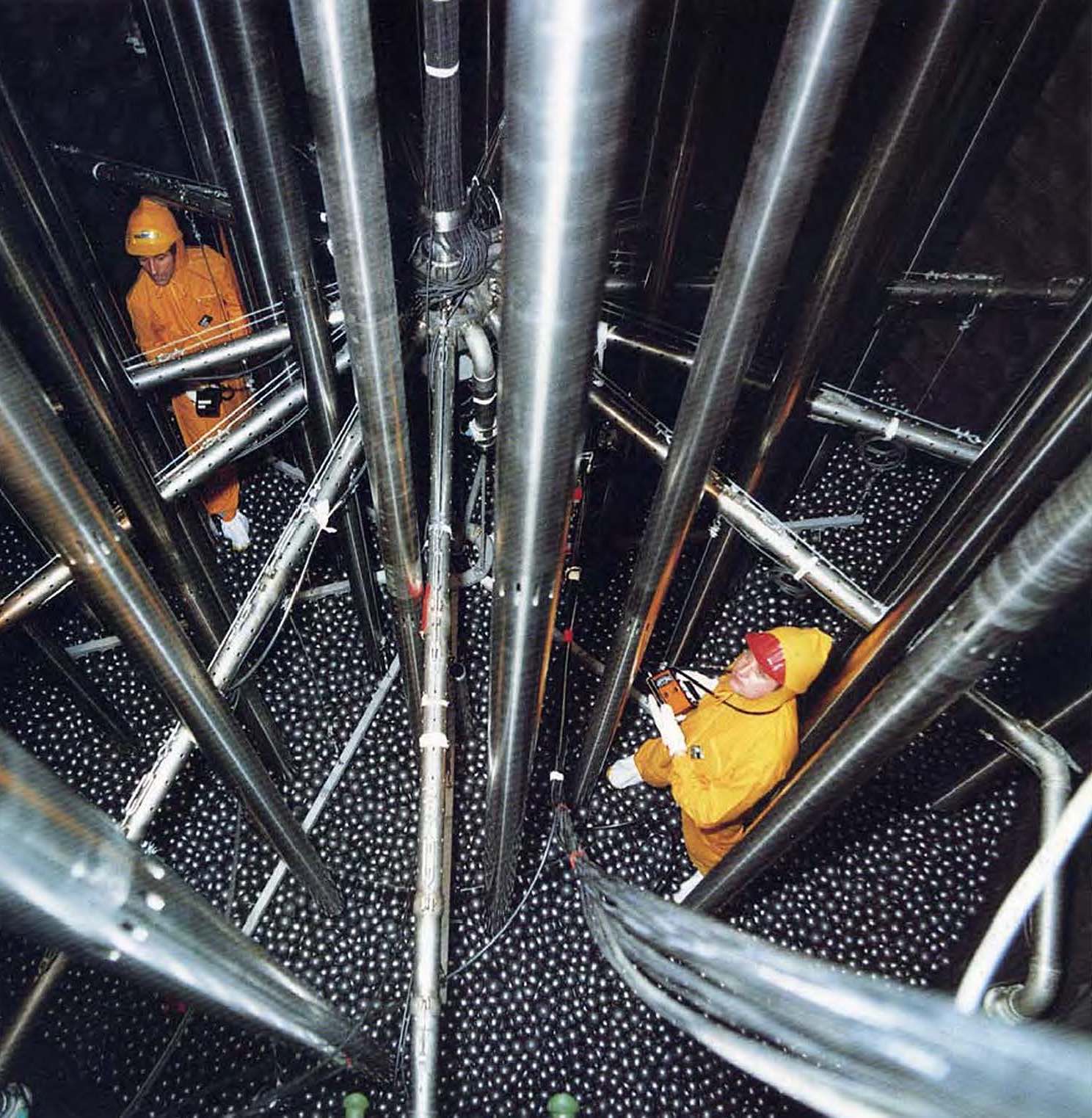
Introduction to Pebble Beds
From its first conception 1 during the 1940s, High Temperature Gas-Cooled Reactors were originally pursued in Germany, the UK, and the USA. Germany focused on pebble-beds with AVR 2 and THTR while the UK and USA focused on prismatic cores with the Peach Bottom Unit 1 and the Ft. Vrain Reactor. After a few demonstrations and in the headwinds from Three Mile Island and Chernobyl, German and American gas-cooled reactor efforts stalled due to lack of customers and government support. Efforts were made to jump-start programs in other countries including South Africa, Japan, and China.
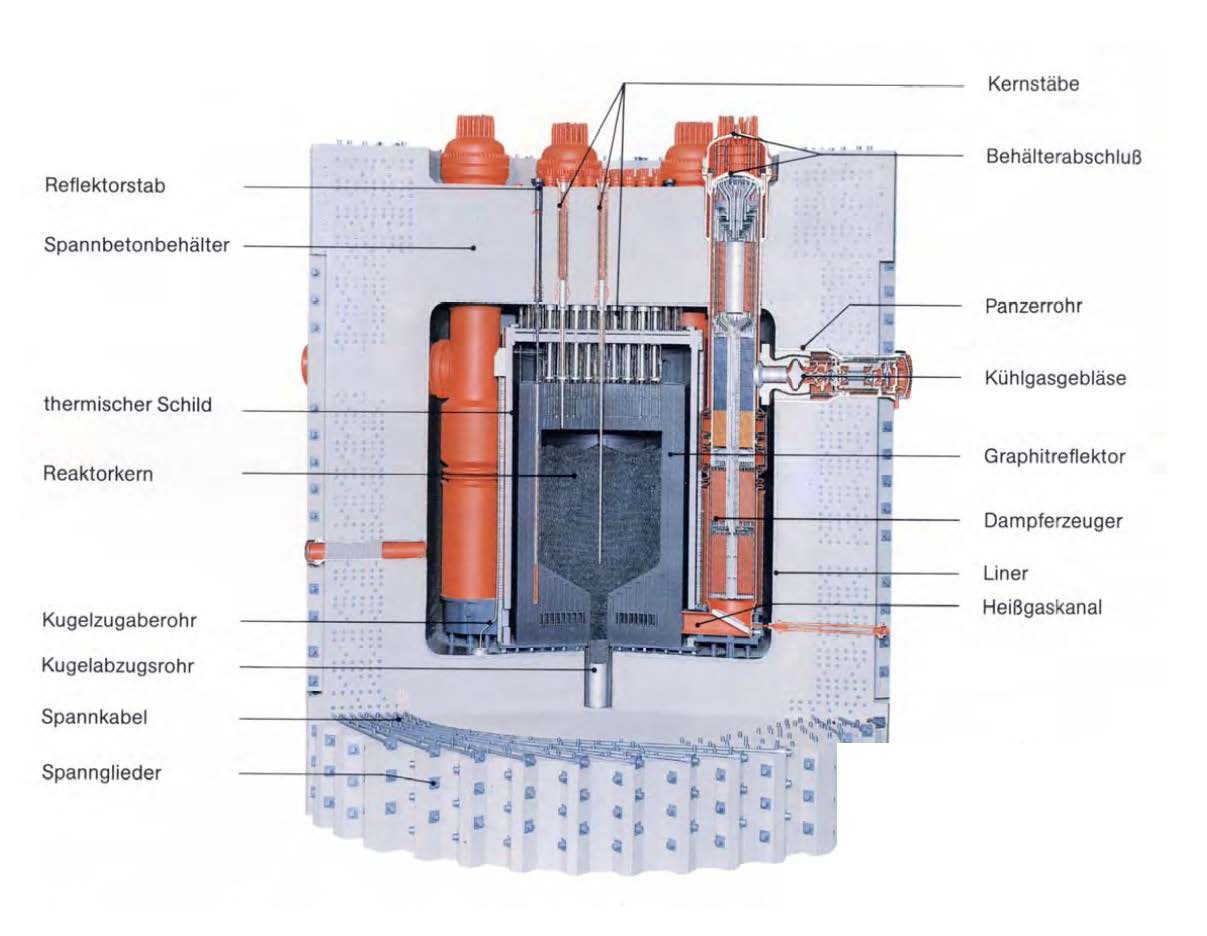
Chinese and South African efforts focused on the pebble-bed approach while Japan pursued prismatic cores. The Chinese began their gas-cooled program in the 1990s with HTR-10, a pebble-bed micro reactor capable of 10 MWe that was operating at full power by 2003. In 2000, China's CNNC began work on the full power demonstrator, HTR-PM, a 250 MWth 105 MWe pebble-bed reactor which achieved full power in 2022. The diagram below shows the Chinese HTR-PM 6-pack, which still uses a large concrete containment structure. The South African Pebble-Bed Modular Reactor (PBMR) was well into development in the 2000s until its primary utility sponsor pulled the plug.3
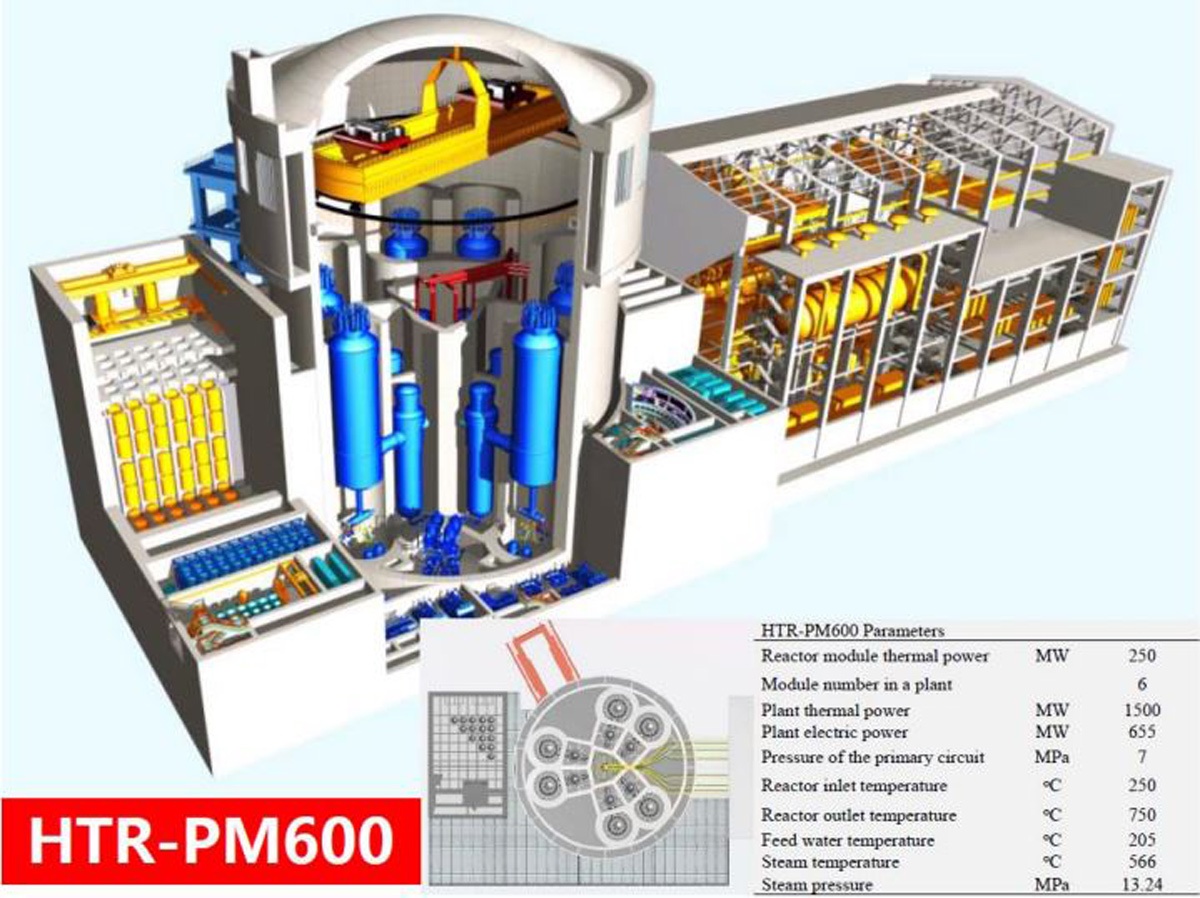
In the US, efforts are being made to develop a pebble-bed similar to the PBMR and the HTR-PM. This Xe-100 is similar to the Chinese HTR-PM in all important aspects, and where it is not the same, it lags behind. For example, the Chinese HTR-PM uses telescoping control rods which reduce the overhead space of the reactor and make it easier to assemble and maintain systems, while the Xe-100 design uses traditional control rods. HTR-PM uses LEU+ (Uranium enriched to less than 9.9%) which is more widely available and not as strictly controlled as Xe-100's 15% HALEU fuel, which has yet to produce fuel as of 2022.
A Continuous Stream of Jostling Pebbles
Fuel Handling / Accounting Challenges
A typical pebble-bed reactor will contain 100,000 – 1,000,000 fuel pebbles in the core at any given time depending on its size. The pebbles come in from the top and drain out the bottom in a constant refueling cycle. Because the reactor is pressurized at 5-10 MPa, inbound pebbles have to go through a compression chamber, and outbound pebbles go through a decompression chamber.
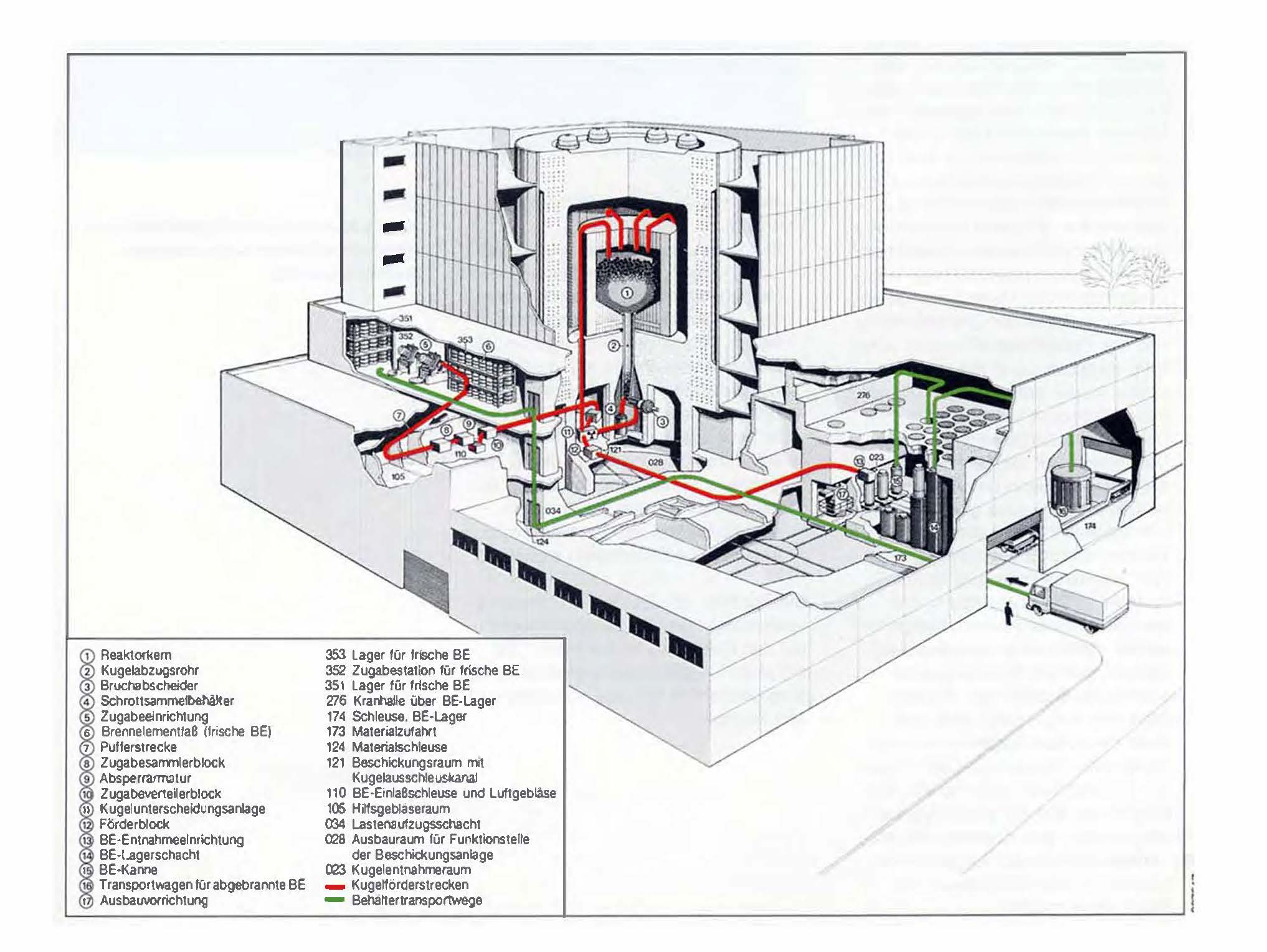
The reactor will discharge roughly a small truckload of pebbles every day. So the power plant site will have to deal with a continuously growing inventory of spent fuel on site and must operate a separate and complex refueling system to maintain the constant stream of pebbles. From the get-go, a pebble-bed customer will need the full fresh and spent fuel handling competence and infrastructure – a costly and challenging capability for most customers to handle and acquire, especially in non-nuclear countries. Accounting for hundreds of thousands of pebbles is not an easy task.
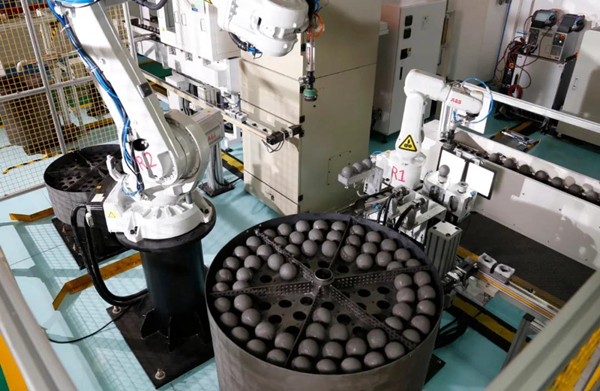
Compare this to prismatic cores that have 2-20 year refueling cycles. In the extreme, MMR has a 20-year core life. The core is placed and sealed, and no new materials are ever introduced into the core. When the core energy is exhausted, the core is replaced with a fresh cartridge in a single refueling event lasting at most a few days. The refueling apparatus will not be site-specific but will be shared across sites. Spent fuel is stored on the site for a limited time before transporting to the repository.
100,000s of moving parts
A pebble-bed will have 100,000s of moving pebbles and 1000s of moving parts to mechanize the pebble feeding and handling infrastructure as well as the control rod systems. This amounts to 100,000s of randomly jostling parts, and 1000s of controlled parts in the primary loop – providing ample opportunity for failure, jams, and maintenance problems. Inevitably, and as in past pebble-beds, some pebbles will crack, release some radioactivity into the primary loop, and jam the feeding system. These types of events require frequent shutdowns and careful unjamming operations, which are not fun in a radioactive environment.
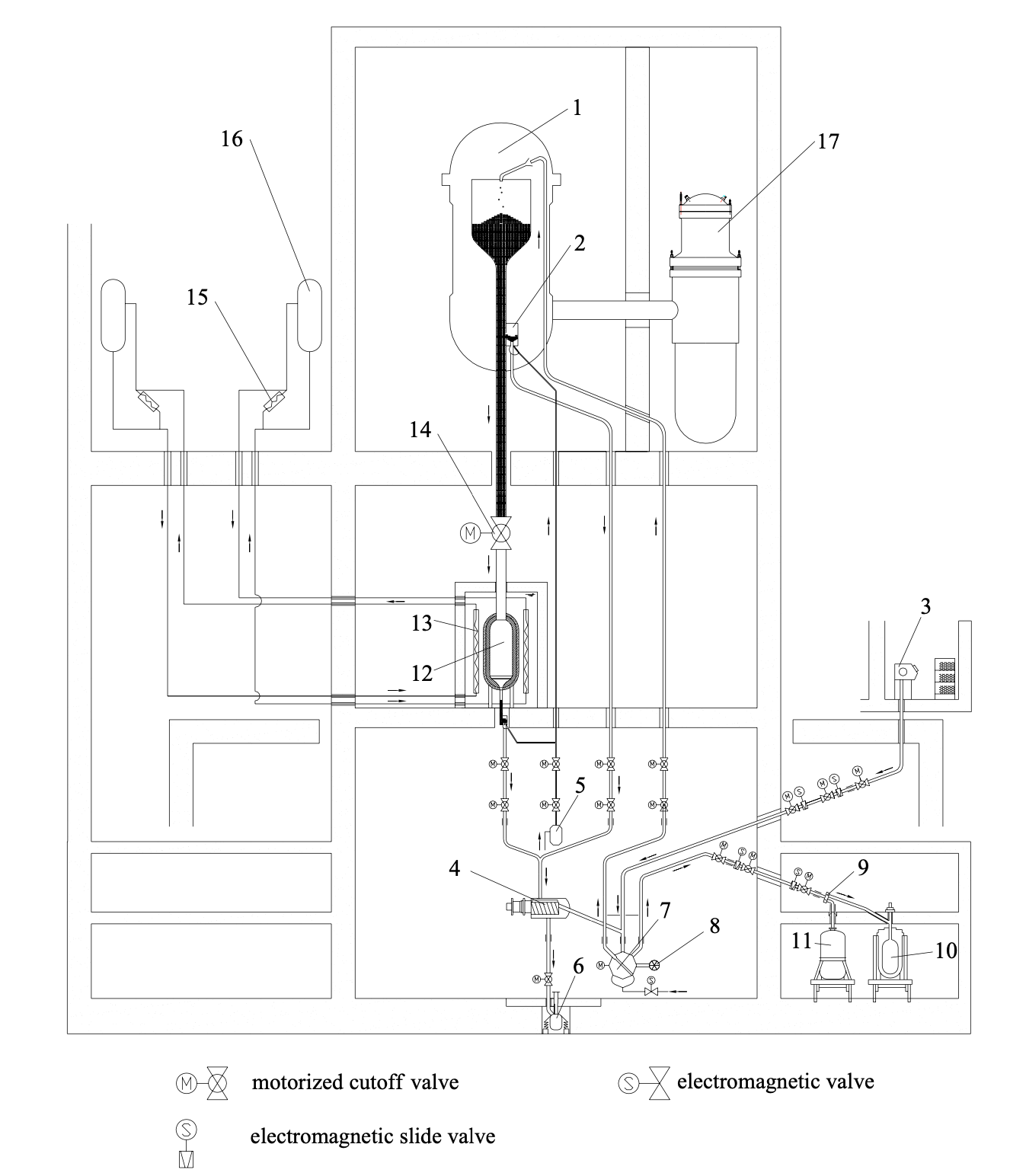
For comparison, a typical prismatic core has about a dozen moving parts, including the control rods, in the primary loop and none of them are strictly necessary to maintain reactor safety.
Proliferation Challenge
Constant refueling offers a continuous opportunity for error such as accidentally introducing incompatible materials in the core. Similarly, bad actors could use custom pebbles to breed their choice of weapon materials. It's a pretty convenient assembly line with fist-sized pebbles of HALEU fuel or breeding material of choice that can be diverted. These proliferation concerns suggest that a HALEU pebble-bed would be a highly controlled nuclear asset (hundreds of security personnel, machine guns, and drones 4) not amenable to worldwide deployments, especially in developing countries that are most in need of new nuclear energy assets but have little nuclear infrastructure or know-how.
A prismatic core's infrequent refueling and couch-sized fuel blocks significantly reduce the proliferation and diversion risk. No new materials are ever introduced into the core once the core is activated. There is no opportunity to breed controlled materials or extract materials. Because prismatic cores are more efficient, they can use lower enriched uranium, including LEU and LEU+, which has an existing supply chain and poses similar diversion targets as current nuclear fuels.
Pebbles limit applications
What happens when the reactor is shaken and the pebbles are quickly reconfigured in new and unknown ways? All the safety analysis and core studies used to license the reactor are not as useful anymore. This could happen during earthquakes or sea motion. The random assortment and free range of motion of the pebbles precludes their use in mobile or marine applications and severely expands the accident analysis space.
Pebble-beds’ single-use moderator = more waste volume and cost
The pebbles have a shell of graphite – that’s how the reactor moderates the neutrons. Because the pebble is one component with combined fuel and moderator, the entire core moderator is being consumed at the same rate as the fuel. For pebble beds, the moderator is as consumable as the fuel, generating larger amounts of fuel-tied waste. Will operators take the risk of separating the moderator and fuel waste? That is extremely doubtful as it risks damaging the fuel and would be a dangerous fuel handling operation.
In a prismatic core, the moderator is used for the entire life of the reactor core and can potentially even be recycled as the moderator and fuel are distinct components.
1-dimensional un-optimizable cores
Pebbles are not good for neutrons. First, packing spheres is volume inefficient. The space between spheres is empty and used to flow coolant. Sphere packing will leave about 30-40% of the core as empty volume. Compare that to the 2-3% of empty space in MMR’s prismatic core. This makes pebble-beds neutronically inefficient compared to prismatic cores, and requires larger reactors to get the same energy and power. That said, the reactor is constantly refueling which allows barely used pebbles from the periphery of the core to be placed in the center of the reactor for higher burnup. Pebble-beds can more easily achieve full burnup of all the fuel compared to a prismatic core with once through fueling.
Beyond the neutronic inefficiencies, all the empty volume in a pebble-bed can lead to unexpected positive reactivity when water fills the voids even at fractions of less than 5%. In other words, the empty space can be filled with water which slows down neutrons and increases the reactivity and power beyond what the reactor is capable of handling.
Perhaps more importantly, a pebble-bed core cannot be designed. It is taken as is, with its inefficiency and wasted volume. On the other hand, a prismatic core can be carefully designed with highly specified geometric configurations that enhance fuel burnups (fraction of the fuel that is burned), flow, and reduce temperatures in the fuel – altogether making for lower cost of energy. Although pebble-beds can reshuffle fuel by taking low burn-up fuel pebbles from the outlet stream and reinserting them closer to the middle of the core, there is virtually no advantage in the final fuel burn-up compared to a prismatic core that can similarly reshuffle fuel blocks.
Because the packing of spheres is random, the cooling configurations are constantly changing in a pebble-bed. This can lead to a high degree of variability and unpredictability in fuel temperatures, with hot spots and insufficient flow. The ultimate result is unexpected fuel failures and coolant contamination. Uncertainty reduces safety.
From 5
First, then, is the issue of pebble bed compaction. A stochastic pebble bed shows a void fraction of 0.4, but the densest possible packing has a void fraction of only 0.26. Pebble flow may lead to compaction, as experiments indicate. Such compaction results in higher temperatures not only because of the higher power density but also because of the significantly larger pressure drop in compacted regions. The pressure drop tends to reduce the coolant flow through compacted regions. Second, fuel elements with high and low burn-up need to be sufficiently mixed over the entire pebble bed. Accumulations of fuel with relatively little burn-up (and high power) raise temperatures significantly. Third, irregularities in pebble flow may change the local heat production and the corresponding core temperature profile. Retardation of fuel element flow, which is unavoidable near reflectors, may lead to unacceptably high burn-up, which enhances fission product release.
In prismatic cores, the coolant channels are simple and fixed over the entire life of the core. There is no randomness. The core is not subject to the jostling and uncertainties of hundreds of thousands of pebbles. The core is a fully 3-D design and can be optimized using high-fidelity modeling and manufacturing tools. For the same reasons, prismatic cores can be much smaller than pebble-bed cores, and find applications in containerized and transportable reactors like Pylon or Kaleidos.
Lower Thermal Conductivity = Less Safe
The empty space in a pebble-bed is not just neutronically wasteful, it's also a safety concern because the thermal conductivity of the core is greatly reduced compared to a solid prismatic core. This means that during accidents, heat remains stuck in the core and piles up, raising temperatures and potentially damaging the fuel.6
Prismatic cores are more thermally conductive and can more easily dissipate excess heat during accidents.
Higher Resistance to Coolant Flows
There is one last drawback to the random pebbles.
The overall coolant flow resistance of a pebble-bed reactor core is inherently greater than the overall coolant flow resistance of a prismatic reactor core. Thus, the core pressure drop will be higher in a pebble-bed reactor than a prismatic reactor for designs having the same core height and coolant flow rates. Consequently, a pebble-bed reactor requires more energy per unit of thermal power output to circulate the coolant, which results in lower pebble-bed reactor plant efficiency.6
Stressing out the Fuel
Another concern for pebble-beds is that the pellets at the bottom must support the entire core’s weight of pebbles above. So now the fuel has a massive structural role in addition to its heat-generating role. It’s already a challenge to manufacture fuel that can contain fission products, but designing fuel that contains fission products AND supports tens of tons of weight AND can survive the jostling impacts of the gumball machine all while being irradiated at high temperatures — now that’s quite the ask! And fuel pebbles often crack and give out, contaminating and jamming the system. In THTR-300, pebbles were cracking at rates 0.5-1.5%, although this was partially due to control rods being in direct contact with fuel pebbles which may no longer be the case with new designs.7 Is this the worst way to use TRISO fuel?
There's also the issue of taking used fuel elements from the bottom of the core and putting them back into the reactor at the top. To do this, a pressure burst imparts an impulse onto pebbles at the bottom so they travel through a tube to the top for sorting, kind of like a pinball machine.
In a prismatic core, the core is structurally supported by hexagonal graphite blocks and there is no jostling or kinetic impacts. The fuel, which is inserted into the graphite blocks, is completely outside of the structural load paths.
Reflector Control Rods
Unlike normal reactors, it appears that Xe-100 only has control rods outside of the core in the reflector. These can only affect leakage neutrons and are less effective than in-core control rods. In-core control rods were used in German THTR, introducing metal tubing in the core, however negating the high temperature safety. In the event of water leakage into the core (40% of which is empty space), as the reactivity of the core increases, neutrons do not leak out of the core as much as normal and the control rods lose effectiveness and other means of control need to be employed, such as perhaps the evacuation of the pebbles from the reactor bed. This was one fundamental reason why General Atomics, when offered to acquire the German Pebble Bed technology in the 1980s, declined to do so. The possibility of water ingress in a pebble bed reactor is a big risk when a high-pressure steam generator is present in the primary circuit, such as for the Xe-100.
The MMR (like all prismatic block reactors) uses in-core control rods which improve the fuel use efficiency and ensure direct reactor control affecting core neutrons across all condition space including water ingress.
Pebble Release
The continuous refueling mechanism requires a system to continuously drain the core of pebbles. In the same way a valve can be used to quickly empty a fluid tank, the pebble bed core is equipped with a system to drain the entire pebble core in the event of accidents. Let me be clear, a pebble-bed is able to drain the core by design. There is then the obvious accident possibility of all the pebbles quickly draining out of the reactor core with potential fractures and leakage of the pressurized loop.
Prismatic cores have no kinetic motion and there is no mechanism for on-line refueling or core draining.
Graphite Dust
The jostling and rubbing of the spheres produces significant amounts of graphite dust that contaminate the coolant and heat transfer components. Graphite dust is a combustible particulate in the presence of oxygen, which occurs anytime the reactor is opened. In the event of coolant leak or air or water ingress, the graphite dust adds a chemical energy component to the accident possibilities. Graphite already poses a fire danger in its bulk form,8 and the dust particles make it worse. Overall, the dust generated in pebble-beds may require extra systems for cleaning and maintenance, and extra accident pathways that are simply not present in a prismatic core.
The quantity of dust in the AVR was a big surprise:
The pebble bed mechanics problems are related to an error made at the beginning of pebble-bed reactor design: it was assumed that the benign lubrication features of graphite are intrinsic properties. Pebble-bed reactors require low friction in the core for smooth pebble flow. However, it was discovered in 1948 (but not sufficiently noticed by AVR designers) that the lubrication behaviour of graphite occurs only in the presence of sufficient humidity, or, in a less efficient manner, in the presence of oxygen. Under the inert conditions required in pebble-bed reactors, the friction coefficient is four times as large, and the wear rate is up to 10,000 times as large. This leads to the formation of large amounts of graphite dust. Unfortunately, all out-of-pile experimental studies on graphite pebble beds were performed under low friction conditions. The major underestimation of graphite friction became obvious after some years of AVR operation because of the large amounts of graphite dust found, because of pebble movement problems in the fuel reshuffling facilities, and because of a pronounced discrepancy between calculated and observed residence time spectra of fuel elements in the core.5
Prismatic cores have no jostling and little to no dust. The surface area of the graphite is 4x lower for a prismatic core relative to a pebble bed, significantly reducing the reaction area and graphite fire risk.
This DOE report came to similar conclusions:
As demonstrated by operating experience in the Fort St. Vrain HTGR and in the AVR, there is much more graphite dust formation in pebble-bed reactors than in prismatic reactors. The circulation of large quantities of graphite dust in the primary coolant loop of pebble-bed reactors has the potential to adversely affect the operation of a direct-cycle power conversion system (PCS) and/or an intermediate heat exchanger (IHX), and could potentially preclude use of printed circuit heat exchangers for the IHX and PCS recuperator. This would be a significant disadvantage for the pebble-bed reactor. Also, the dust is an excellent medium for enhanced release of fission products during accidents involving depressurization of the primary coolant loop. Indeed, the quantity of graphite dust that would be expected in the primary circuit of the PBMR-400 based on AVR experience raises a question as to whether a PBMR-400 with a VLPC can meet off-site dose limits (assuming a 425-m plant exclusionary boundary) during a rapid depressurization accident.6
Reflector Reliance
Because of the empty volume in the core, pebble beds have to use very big graphite reflectors to slow down and reflect neutrons back into the core. And to be cost competitive, that reflector has to survive 20 years' worth of damage at very high power ratings. The problem is that graphite is not likely to withstand this kind of damage.
Acceptable graphite performance to a fast neutron fluence of this magnitude has not been demonstrated, and qualification of graphite to such high fast neutron fluence will be problematic. Consequently, the graphite reflectors in a pebble-bed reactor annular core design represent a significant design risk. More frequent reflector replacement, should this be necessary, would have a significant impact on pebble-bed reactor plant availability because replacement of the pebble-bed reactor reflectors is estimated to require an approximate 6 month outage. (In contrast, the non-permanent reflector blocks in a prismatic reactor can be replaced during the normal periodic refueling outages.)6
Design Dead End
Perhaps the biggest limitation of a pebble-bed framework is the inability to design and control the core and improve the core design in future generations. The designer is more or less stuck in an evolutionary cul-de-sac with a bucket of pebbles - randomly packed and inefficient, draining out of the core however they want. The design cannot control enrichment or moderation across the core and has to waste 30-40% of the volume on empty space. They can’t put variable moderation or cooling channels. They can’t directly cool the fuel which helps improve burn-ups and fuel efficiency and reduces stresses on the moderator. The pebble-bed is stuck with limited control systems that take up significant core volume. There is little to no opportunity to design and improve core performance.
The future of core design is using highly specified coolant channels that vary across the core, highly specified fuel dimensions and enrichments, and the use of new and far more efficient moderators. These technologies and methods are only available in a prismatic HTGR framework, using advanced manufacturing of ceramics and algorithmic/AI design methods. Prismatic HTGRs are the enabler for highly fuel-efficient and compact cores that minimize the cost of energy.
Brief not on the Fluoride Salt-Cooled High-Temperature Reactor
The Fluoride Salt-Cooled High-Temperature Reactor (FHR) are reactors that use a graphite moderator, solid fuels, and a molten-salt coolant. The most promising versions of the FHR use TRISO particle fuel and fluoride-salt coolant. Kairos Power is the major developer for FHRs in the USA.
Utilizing TRISO Fuel and Fluoride Salt Coolant
Fluoride-salt reactors are expected to use TRISO fuel—known for its stability at very high temperatures—and low-pressure fluoride salt as a coolant instead of conventional water. An FHR can be designed either as a pebble-bed core or a prismatic core, both employing graphite moderator and reflector blocks.
In a pebble-bed FHR, pebbles incorporate an internal cavity or low-density graphite core so that they float in a pool of molten fluoride salt. Pebbles are fed in from the bottom and float upward through the core. They can contain TRISO particle fuel; when the pebble uses a silicon-carbide matrix, it is called Fully Ceramic Micro-encapsulated (FCM) fuel. Molten fluoride salts enable efficient heat transfer at high temperatures and effectively trap fission products. Studies show these salts are compatible with high-temperature structural materials such as stainless steel, supporting reliable service life and commercial viability.
Low Pressure and Online Refueling
An FHR operates at low pressure, reducing the need for thick-walled pressure vessels or high-pressure containment structures during normal operation. Because fuel pebbles circulate continuously, the reactor can be refueled online, minimizing downtime and improving operational efficiency. Past experience, however, shows that moving pebbles can pose challenges—including graphite dust generation, coolant-flow blockages, and fuel cracking.
FHR technology thus offers an alternative pathway for clean-energy generation, blending high safety margins, reliable performance, and favorable economics while leveraging the robust TRISO particle fuel design.
Conclusions
Pebble-beds are an obsolete design of the 1960s that did not progress well and don’t solve many of the nuclear industry’s problems: manufacturing, cost, complexity, safety, and scaling. Many of the old-nuclear problems are made worse by the pebble-bed’s design constraints. Even in China, now the world leader in pebble-bed technology with multiple operating units, it's not clear that further pebble-beds will be built.
The circulating ball operation scheme requires a complex, continuous, and jamming-prone refueling activity. The consumable moderator requires a large spent fuel infrastructure. The ability to refuel on-line is NOT an advantage, and introduces concerns of proliferation and diversion and the need for constant on-site surveillance of operations at least as comprehensive as current Light Water Reactors (LWRs) and CANDUs.
Even the US DOE concluded that pebble-beds were not likely to be effective power reactors and would present between 10% and 20% worse economics than prismatic reactors.
The overall conclusion of the current pebble-bed reactor vs. prismatic reactor comparison is that the prismatic has a clear advantage over the pebble-bed as the modular helium reactor type best suited for a commercial very high temperature reactor (VHTR) for electricity production and various high-temperature process heat applications, including hydrogen production.6
It's very strange that DOE has now reversed course to fund the Xe-100 to the tune of several billion dollars as part of the Advanced Reactor Demonstration Project. This flies in the face of German reactor experience, the failed South African PBMR project 3, and the lack of commercial plans coming from China's pebble-bed demonstrations.
Pebble-beds will be built in ones or twos, but may not be a good choice for deployments of hundreds or thousands of units. There are simply too many other better options, and I predict that the pebble-bed developers will either go under or transition to prismatic designs.
Various images from Kerntechnische Gesellschaft (KTG)
Footnotes
-
Thomas, The Pebble Bed Modular Reactor: An obituary, 2011 ↩ ↩2
-
Xe-100 White Paper: Physical Protection System Approach Revision ↩
-
Moormann report: Pebble-bed Reactor Safety Revisited (pdf), 2009 ↩ ↩2
-
DOE Report NGNP Reactor Type Comparison Study Report, 2007 ↩ ↩2 ↩3 ↩4 ↩5
-
Moormann, Phenomenology of Graphite Burning in Air Ingress Accidents of HTRs, 2011 ↩